One of the principal marine environmental problems associated with climate change is ocean deoxygenation (loss of oxygen). Deoxygenation has been observed in most global oceans in recent decades, according to experimental data. Furthermore, earth system models predict that oxygen concentrations in most of the world’s oceans will decrease in the future.
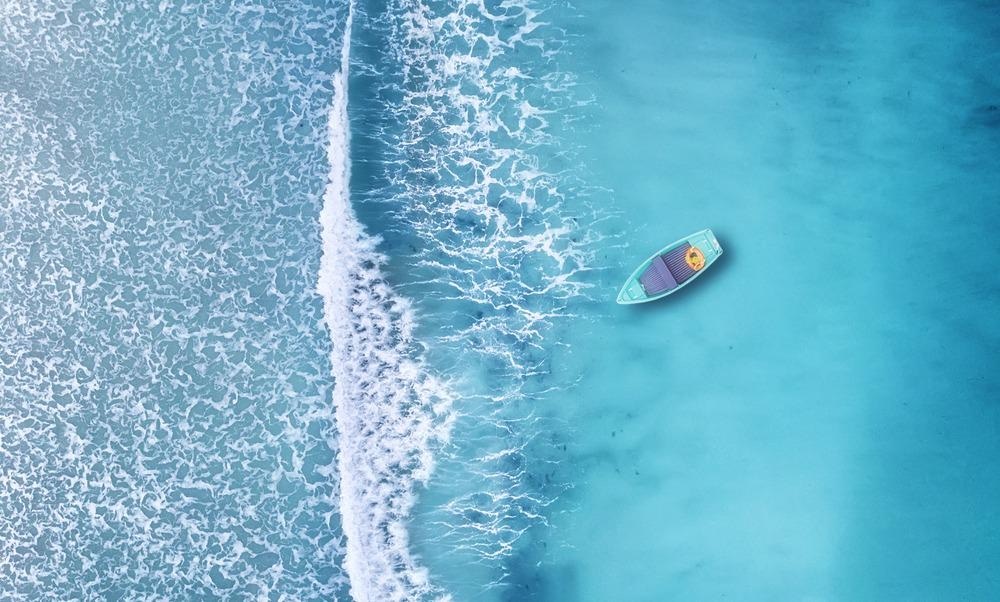
Image Credit: biletskiyevgeniy.com/Shutterstock.com
Deoxygenation boosts greenhouse gas emissions, inhibits diverse life processes, diminishes halobiotic abundance, and collapses ecosystems.
Caused by global warming, ocean deoxygenation indicators may outperform their internal variability as the temperature rises. In the absence of external forcing, the time of emergence (ToE) is used to determine when a climate variable’s indication of change arises from its internal variability.
A Breathless Ocean
Video Credit: IUCN, International Union for Conservation of Nature/YouTube.com
Researchers looked at the ToE of oxygen changes over all vertical zones of the global ocean as predicted by two sets of large ensemble simulations from the Common Earth System Model (CESM) that represented low- and high-emission paths. Their research was published in Geophysical Research Letters.
Methodology
Researchers used data from 40 ensemble members of the Community Earth System Model Large Ensemble Numerical Simulation (CESM-LENS) project to calculate monthly dissolved oxygen (mmol∙m−3) and surface air temperature (°C).
To acquire reliable estimates of the signals of oceanic oxygen shift in response to climate warming, a pattern scaling methodology was employed. The first year when the signal of a continual drop in oxygen concentration surpasses the internal variability is characterized as the ToE of deoxygenation.
To prevent a possible brief exceedance signal that is unknown if the projected ToE is close to the end of the data, researchers only concentrated on the ToE that is at least 20 years earlier than the end of the time series in this investigation.
The ToEs of deoxygenation in each layer were computed first since there were 60 vertical layers. By averaging the ToE estimations over the layers inside each zone, the epipelagic, mesopelagic, and bathypelagic ToEs were calculated. The standard deviation of the estimations in all layers inside this zone equaled the ToEs’ equivalent uncertainty.
Figure 1 shows that deoxygenation occurs in more locations of the global ocean from 1920 to 2100, with a higher change magnitude than oxygenation, under the RCP 8.5 scenario.
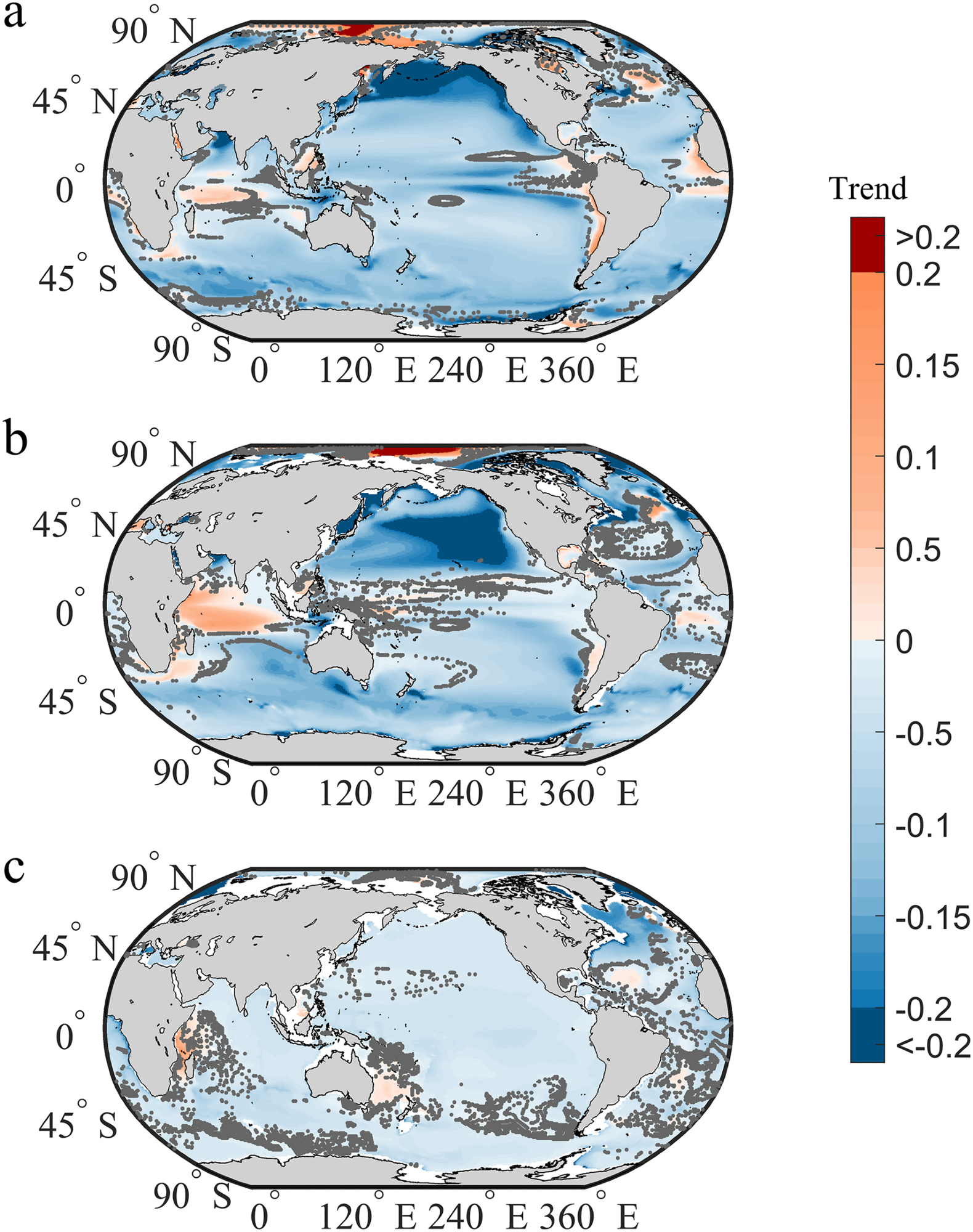
Figure 1. Global dissolved oxygen changes (mmol m−3 per year) from 1920 to 2100 under the RCP8.5 scenario. The linear trends of oceanic oxygen changes are estimated in the (a) epipelagic, (b) mesopelagic, and (c) bathypelagic zones. The blue and red colors represent deoxygenation and oxygenation, respectively. Note that the dark gray points indicate the slopes are not statistically significant at p = 0.05 level. Image Credit: Gong, et al. 2021
Except for some coastal oceans, tropical oceans, and the northern North Atlantic, deoxygenation signs would appear in most ocean areas by 2080 (Figures 2a–2c).
The correlating deoxygenation signals are predicted to arise before 2030 in the mesopelagic zone of the North Pacific, the southern Atlantic Ocean, and the high-latitude (>45° S) southern oceans, which is anticipated to encounter deoxygenation across 100% of the vertical layers in this zone (Figures 2a–2f).
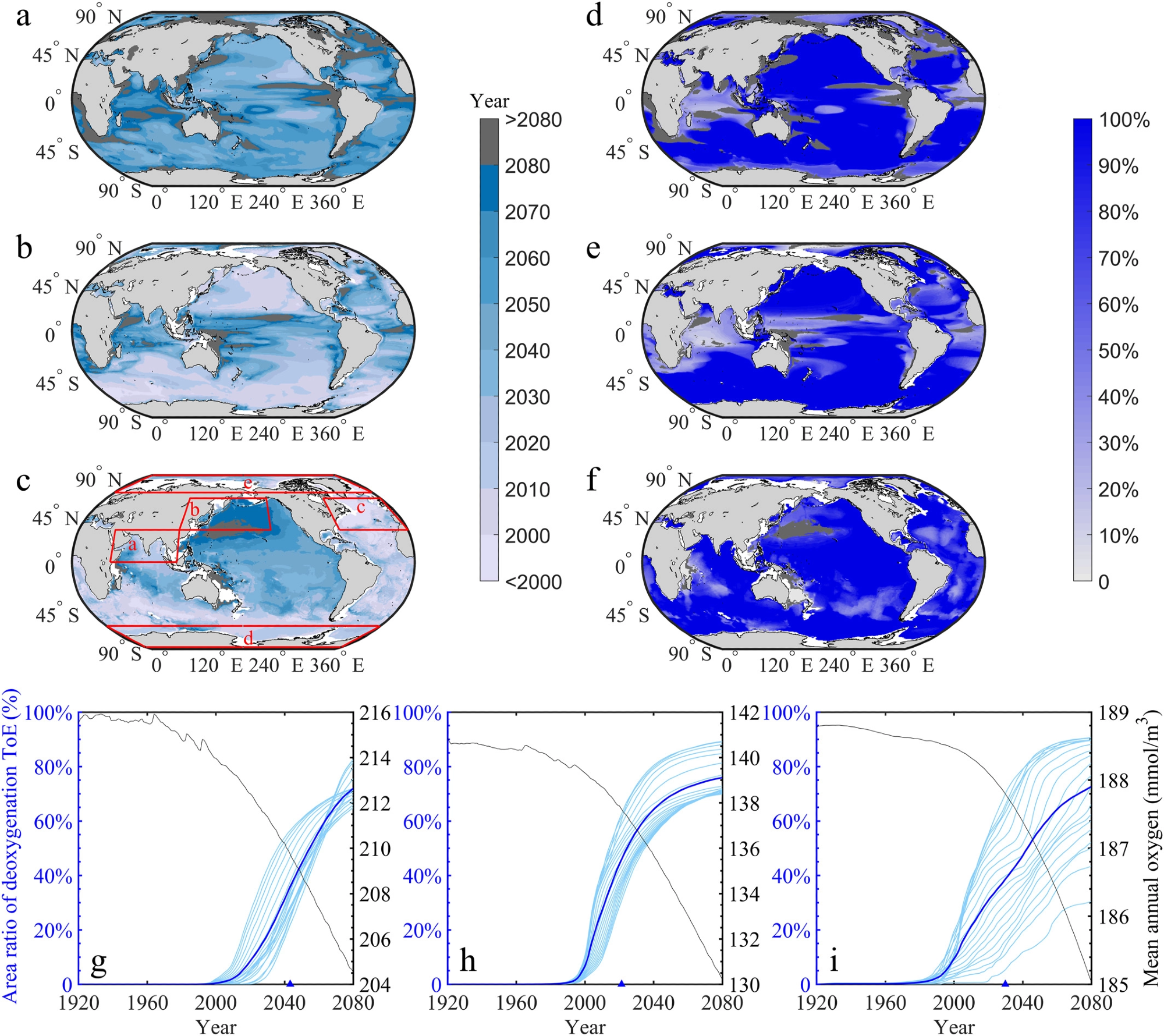
Figure 2. Time of emergences (ToEs) of the deoxygenation signal in the epipelagic, mesopelagic, and bathypelagic zones under the RCP8.5 scenario from 1920 to 2080. Spatial maps show the mean ToEs of all the deoxygenation layers within each zone (a–c), and the percentage of the vertical layers that are projected to experience deoxygenation within each zone at each grid (d–f). The deoxygenation ratio of each zone represents the fraction of the layers that are characterized by deoxygenation to all layers within that zone. Dark gray indicates no emergence of deoxygenation by 2080. The right y-axis of the bottom panel shows a time series of global oxygen concentrations (black curve) of the (g) epipelagic, (h) mesopelagic, and (i) bathypelagic zones. The left y-axis shows cumulative horizontal coverage ratios of all layers (light blue curves) within a zone (dark blue curves) where the deoxygenation signals emerge. The cumulative horizontal coverage ratio of the deoxygenation signal is the ratio of the area with deoxygenation signals in the oceanic area (g–i). Horizontally, the spatial coverage ratio of the deoxygenation signal is the ratio of the area with the deoxygenation signal to the oceanic area (g–i). The global mean ToEs of the (g) epipelagic, (h) mesopelagic, and (i) bathypelagic zones are indicated by blue triangles. Image Credit: Gong, et al. 2021
The global mean oxygen concentration in the mesopelagic, epipelagic, and bathypelagic zones, is illustrated in Figure 3. As a result, the dropping trends in these locations are not significant (Figure 1b), and the ToE occurs reasonably early (Figure 2c), given the minimal internal variation.
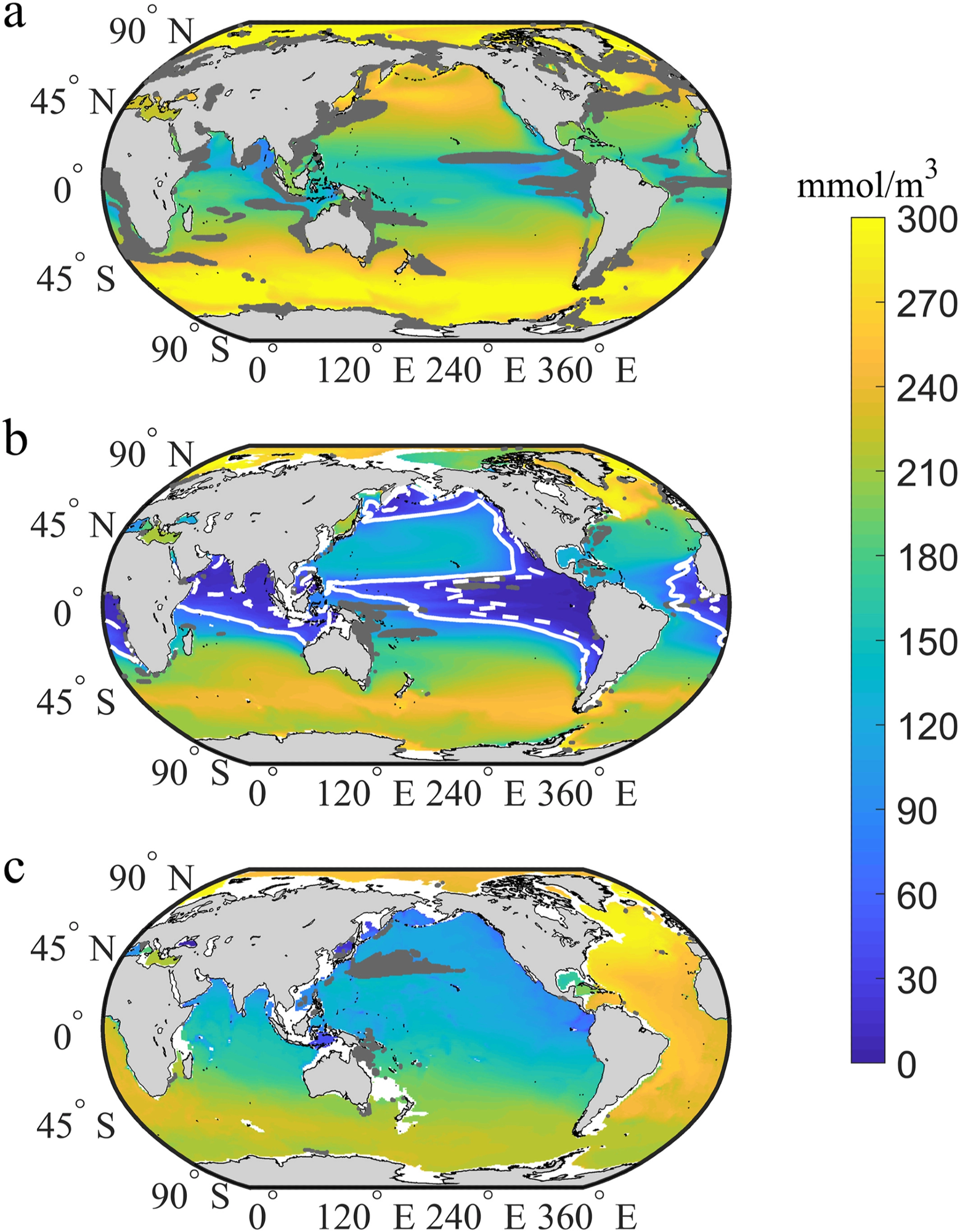
Figure 3. Distribution of oxygen concentrations at the time of emergence (O2ToE) in the (a) epipelagic, (b) mesopelagic, and (c) bathypelagic zones under the RCP8.5 scenario. The white solid and dashed lines represent the oxygen concentrations of 20 and 60 mmol/m3, respectively. Dark gray indicates no emergence of deoxygenation by 2080. Image Credit: Gong, et al. 2021
Researchers worked on five sample oceanic regions to learn more about ToE characteristics in regional oceans: the North Indian Ocean, Southern Ocean, North Atlantic Ocean, Western North Pacific Ocean, and Arctic Ocean (Figure 2c). Among the three vertical zones in the North Indian Ocean, the bathypelagic zone is expected to see the fastest and most extensive appearance of the deoxygenation indication (Figures 4a–4c).
Furthermore, from 2040 to 2080, the mean oxygen content in the mesopelagic zone indicates a considerable upward trend. Despite the fact that oxygen concentrations in the ocean continue to rise during this time, mesopelagic oxygen concentrations remain consistently below 15 mmol m−3 (Figure 4b).
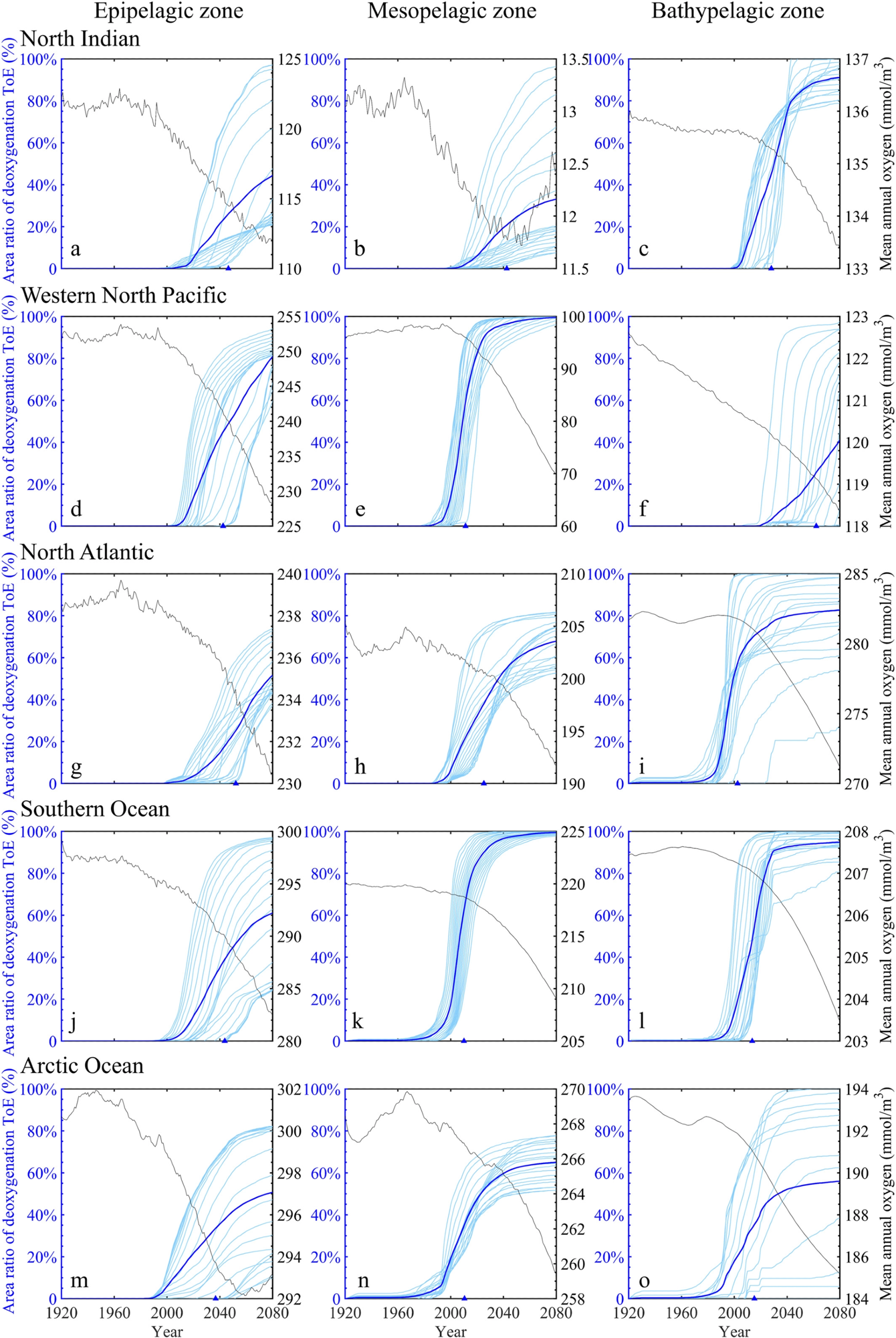
Figure 4. Time series of regional oxygen concentrations, cumulative horizontal coverage ratios where deoxygenation signals emerge and mean time of emergences (ToEs) for all layers within the epipelagic, mesopelagic, and bathypelagic zones of the selected ocean regions, including the North Indian (a–c; 0–30° N, 40–110° E), western North Pacific (d–f; 30–60° N, 110° E−150° W), Southern (g–i; 60–85°S), the North Atlantic (j–l; 30–60° N, 75° W–0° W), and Arctic Oceans (m-o; >66° N). The zonal mean ToEs of the regional epipelagic, mesopelagic, and bathypelagic zones are indicated in blue triangles. Image Credit: Gong, et al. 2021
Early-onset of deoxygenation with extensive spatial coverage is predicted in the mid-to-high-latitude oceans, particularly below the epipelagic zone (Figure 4d–4l). Before 2080, almost the whole mesopelagic zone in the western North Pacific Ocean has a deoxygenation signal, with an average ToE of 2011 (Figure 4e).
Deoxygenation signals appear in 99% of the Southern Ocean’s mesopelagic zone and 95% of the Southern Ocean’s bathypelagic zone by 2080 (Figures 4k–4l). The deoxygenation signal appears sooner in the deeper layers (Figures 4g–4i).
In the Arctic Ocean, the mesopelagic zone has the most spatial coverage of deoxygenation signals, and the mean ToE is the earliest of the three vertical zones (Figures 4m–4o).
Results and Discussion
Under the RCP8.5 scenario, deoxygenation signals would appear in the epipelagic, mesopelagic, and bathypelagic zones before 2050. Deoxygenation ToEs would happen in more than 72% of ocean regions in each zone by 2080. Rising temperatures or weakening meridional overturning circulations caused by climate change are the main causes of extensive deoxygenation.
Due to the bigger temperature variations between vertical zones, the epipelagic zone warms quickly when compared to the mesopelagic and bathypelagic zones, making ocean stratification more robust. Ocean stability prohibits epipelagic oxygen from reaching the other two zones.
Furthermore, as meridional overturning circulations weaken, epipelagic oxygen entrance into the two bottom zones is limited, leading to lower oxygen concentrations in the mesopelagic and bathypelagic zones.
The early appearance of deoxygenation signals with broad spatial coverage in the mesopelagic and bathypelagic zones is expected in the mid-to high-latitude seas, such as the western North Pacific, North Atlantic, and Southern Oceans, before 2080.
Conclusion
This research demonstrates the temporal restrictions of variations in oxygen concentration from the ocean’s top to its depths, as well as the degree of deoxygenation in marine ecosystems as a result of climate change. Such information is useful in the development of maritime environmental management policies.
Early signs of deoxygenation can have an effect on marine life and resources. The mesopelagic zone is a crossroads for upwelling currents and the primary habitat for a variety of commercial fisheries, as well as a key player in geochemical circulation. More research is needed to evaluate the possible reductions in biodiversity of deoxygenation-sensitive fishes.
Journal Reference:
Gong, H., Li, C., Zhou, Y. (2021) Emerging global ocean deoxygenation across the 21st century. Geophysical Research Letters, 48(23), p. e2021GL095370. Available Online: https://agupubs.onlinelibrary.wiley.com/doi/10.1029/2021GL095370.
References and Further Reading
- Andreev, A & Watanabe, S (2002) Temporal changes in dissolved oxygen of the intermediate water in the subarctic North Pacific. Geophysical Research Letters, 29(14), pp. 25–31. doi.org/10.1029/2002GL015021.
- Bilbao, R. A. F., et al. (2015) Analysis of the regional pattern of sea level change due to ocean dynamics and density change for 1993–2099 in observations and CMIP5 AOGCMs. Climate Dynamics, 45(9), pp. 2647–2666. doi.org/10.1007/s00382-015-2499-z.
- Bopp, L., et al. (2013) Multiple stressors of ocean ecosystems in the 21st century: Projections with CMIP5 models. Biogeosciences, 10(10), pp. 6225–6245. doi.org/10.5194/bg-10-6225-2013.
- Bruno, J. F., et al. (2018) Climate change threatens the world’s marine protected areas. Nature Climate Change, 8(6), pp. 499–503. doi.org/10.1038/s41558-018-0149-2.
- Cartapanis, O., et al. (2011) Northeastern Pacific oxygen minimum zone variability over the past 70 kyr: Impact of biological production and oceanic ventilation. Paleoceanography, 26 (4). doi.org/10.1029/2011PA002126.
- Costello, M J & Breyer, S (2017) Ocean depths: The mesopelagic and implications for global warming. Current Biology, 27(1), pp. R36–R38. doi.org/10.1016/j.cub.2016.11.042.
- Davison, P. C., et al. (2013) Carbon export mediated by mesopelagic fishes in the northeast Pacific Ocean. Progress in Oceanography, 116, pp. 14–30. doi.org/10.1016/j.pocean.2013.05.013.
- Del Raye, G &Weng, K C (2015) An aerobic scope-based habitat suitability index for predicting the effects of multi-dimensional climate change stressors on marine teleosts. Deep-Sea Research Part II-Topical Studies in Oceanography, 113, pp. 280–290.doi.org/10.1016/j.dsr2.2015.01.014
- Diffenbaugh, N S & Scherer, M (2011) Observational and model evidence of global emergence of permanent, unprecedented heat in the 20th and 21st centuries. Climatic Change, 107(3), pp. 615–624. doi.org/10.1007/s10584-011-0112-y.
- Emerson, S., et al. Temporal trends in apparent oxygen utilization in the upper pycnocline of the North Pacific: 1980-2000, Journal of Oceanography, 60(1), pp. 139–147. doi.org/10.1023/B:JOCE.0000038323.62130.a0.
- Froelicher, T. L., et al. (2009) Natural variability and anthropogenic trends in oceanic oxygen in a coupled carbon cycle-climate model ensemble. Global Biogeochemical Cycle, 23. doi.org/10.1029/2008gb003316.
- Frölicher, T. L., et al. (2016) Sources of uncertainties in 21st century projections of potential ocean ecosystem stressors. Global Biogeochemical Cycle, 30(8), pp. 1224–1243. doi.org/10.1002/2015gb005338.
- Gruber, N (2011) Warming up, turning sour, losing breath: Ocean biogeochemistry under global change. Philosophical Transactions of the Royal Society of A, 369, pp. 1980– 1996. doi.org/10.1098/rsta.2011.0003.
- Hameau, A., et al. (2020) Isdeoxygenation detectable before warming in the thermocline? Biogeosciences, 17(7), pp. 1877–1895. https://doi.org/10.5194/bg-17-1877-2020.
- Hameau, A., et al. (2019) Assessment of time of emergence of anthropogenic deoxygenation and warming: Insights from a CESM simulation from 850 to 2100 CE. Biogeosciences, 16(8), pp. 1755–1780. doi.org/10.5194/bg-16-1755-2019.
- Hawkins, E., et al. (2014) Uncertainties in the timing of unprecedented climates. Nature, 511(7507), pp. E3–E5. doi.org/10.1038/nature13523.
- Hawkins, E., et al. (2020) Observed emergence of the climate change signal: From the familiar to the unknown. Geophysical Research Letters, 47(6), p. 10. https://doi.org/10.1029/2019gl086259.
- Hawkins, E & Sutton, R (2012) Time of emergence of climate signals. Geophysical Research Letters, 39, p. 6. https://doi.org/10.1029/2011gl050087.
- Helm, K. P., et al. (2011) Observed decreases in oxygen content of the global ocean. Geophysical Research Letters, 38, p. L23602. doi.org/10.1029/2011gl049513.
- Henson, S. A., et al. (2017) Rapid emergence of climate change in environmental drivers of marine ecosystems. Nature Communications, 8, p. 9. doi.org/10.1038/ncomms14682.
- Irigoien, X., et al. (2014) Large mesopelagic fishes biomass and trophic efficiency in the open ocean. Nature Communications, 5, p. 10. doi.org/10.1038/ncomms4271.
- Ito, T., et al. (2017) Upper ocean O2 trends: 1958–2015. Geophysical Research Letters, 44(9), pp. 4214–4223. doi.org/10.1002/2017gl073613.
- Jaccard, S. L., et al. (2014) Ocean (de)oxygenation across the last glaciation. Oceanography, 27(1), pp. 26–35. https://doi.org/10.5670/oceanog.2014.05.
- Kay, J. E., et al. (2015). The community earth system model (CESM) large ensemble project: A community resource for studying climate change in the presence of internal climate variability. Bulletin of the American Meteorological Society, 96(8), pp. 1333– 1349. https://doi.org/10.1175/bams-d-13-00255.1.
- Keeling, R. F., et al. (2010). Ocean Deoxygenation in a Warming World. In. C. A. Carlson, & S. J. Giovannoni (Eds.), Annual review of marine science (Vol. 2, pp. 199– 229). Annual Reviews. https://doi.org/10.1146/annurev.marine.010908.163855.
- King, A. D., et al. (2015) The timing of anthropogenic emergence in simulated climate extremes. Environmental Research Letters, 10(9), p. 9. doi.org/10.1088/1748-9326/10/9/094015.
- King, A. D., et al. (2017) Timing of Anthropogenic emergence in climate extremes. In. Climate extremes. pp. 93–103. doi.org/10.1002/9781119068020.ch6.
- Kock, A., et al. (2016) Extreme N2O accumulation in the coastal oxygen minimum zone off Peru. Biogeosciences, 13(3), pp. 827–840. doi.org/10.5194/bg-13-827-2016.
- Lachkar, Z., et al. (2018) Intensification and deepening of the Arabian Sea oxygen minimum zone in response to increase in Indian monsoon wind intensity. Biogeosciences, 15 (1), pp. 159–186. doi.org/10.5194/bg-15-159-2018.
- Levin, L A (2018) Manifestation, Drivers, and Emergence of Open Ocean Deoxygenation. In. C. A. Carlson, & S. J. Giovannoni (Eds.), Annual review of marine Science, 10, pp. 229–260. Annual Reviews. doi.org/10.1146/annurev-marine-121916-063359
- Li, G., et al. (2020) Increasing ocean stratification over the past half-century. Nature and Climate Change, 10(12), pp. 1116–1123. doi.org/10.1038/s41558-020-00918-2.
- Liu, W., et al. (2020) Climate impacts of a weakened Atlantic Meridional Overturning Circulation in a warming climate. Science Advances, 6(26), p. 8. https://doi.org/10.1126/sciadv.aaz4876.
- Long, M. C., et al. (2016) Finding forced trends in oceanic oxygen. Global Biogeochemical Cycle, 30(2), pp. 381–397. doi.org/10.1002/2015gb005310.
- Luyten, J. R., et al. (1983) The ventilated thermocline. Journal of Physical Oceanography, 13(2), pp. 292–309. doi.org/10.1175/1520-0485(1983)013<0292:Tvt>2.0.Co;2.
- Lyu, K., et al. (2014) Time of emergence for regional sea-level change. Nature Climate Change, 4(11), pp. 1006–1010. doi.org/10.1038/nclimate2397.
- Maroon, E. A., et al. (2018) Influence of the Atlantic Meridional overturning circulation on the Northern Hemisphere surface temperature response to radiative forcing. Journal of Climate, 31(22), pp. 9207–9224. https://doi.org/10.1175/jcli-d-17-0900.1.
- McCormick, L R & Levin, L A (2017) Physiological and ecological implications of ocean deoxygenation for vision in marine organisms. Philosophical Transactions of the Royal Society of A-Maths Physics Engineering Sciences, 375(2102), p. 26. doi.org/10.1098/rsta.2016.0322.
- Nakanowatari, T., et al. (2007) Warming and oxygen decrease of intermediate water in the northwestern North Pacific, originating from the Sea of Okhotsk, 1955–2004. Geophysical Research Letters, 34(4). doi.org/10.1029/2006GL028243.
- Oschlies, A., et al. (2008) Simulated 21st century's increase in oceanic suboxia by CO2-enhanced biotic carbon export. Global Biogeochemical Cycle, 22(4). doi.org/10.1029/2007GB003147.
- Paulmier, A & Ruiz-Pino, D (2009) Oxygen minimum zones (OMZs) in the modern ocean. Progress in Oceanography, 80(3–4), pp. 113–128. doi.org/10.1016/j.pocean.2008.08.001.
- Rixen, T., et al. (2020) Present past and future of the OMZ in the northern Indian Ocean. Biogeosciences Discussion, pp. 1–46. doi.org/10.5194/bg-2020-82.
- Rodgers, K. B., et al. (2015) Emergence of multiple ocean ecosystem drivers in a large ensemble suite with an Earth system model. Biogeosciences, 12(11), pp. 3301–3320. doi.org/10.5194/bg-12-3301-2015.
- Sayre, R. G., et al. (2017) A three-dimensional mapping of the ocean based on environmental data. Oceanography, 30(1), pp. 90–103. doi.org/10.5670/oceanog.2017.116.
- Schmidtko, S., et al. (2017) Decline in global oceanic oxygen content during the past five decades. Nature, 542(7641), pp. 335–339. doi.org/10.1038/nature21399.
- Silvy, Y., et al. (2020) Human-induced changes to the global ocean water masses and their time of emergence. Nature Climate Change, doi.org/10.1038/s41558-020-0878-x.
- Stramma, L., et al. (2008) Expanding oxygen-minimum zones in the tropical oceans. Science, 320(5876), pp. 655–658. doi.org/10.1126/science.1153847.
- Sui, Y., et al. (2014) Time of emergence of climate signals over China under the RCP4.5 scenario. Climatic Change, 125 (2), pp. 265–276. doi.org/10.1007/s10584-014-1151-y.
- Takano, Y., et al. (2018) Projected centennial oxygen trends and their attribution to distinct ocean climate forcings. Global Biogeochemical Cycles, 32(9), pp. 1329–1349. doi.org/10.1029/2018GB005939.
- Talley, L. D., et al. (2016) Changes in ocean heat, carbon content, and ventilation: A review of the first decade of GO-SHIP global repeat hydrography. Annual Reviews of Marine Sciences, 8(1), pp. 185–215. doi.org/10.1146/annurev-marine-052915-100829.
- Tjiputra, J. F., et al. (2018) Mechanisms and early detections of multidecadal oxygen changes in the interior subpolar North Atlantic. Geophysical Research Letters, 45(9), pp. 4218–4229. doi.org/10.1029/2018gl077096.
- Vedor, M., et al. (2021) Climate-driven deoxygenation elevates fishing vulnerability for the ocean's widest ranging shark. eLife, 10. doi.org/10.7554/eLife.62508.
- Watson, A. J., et al. (2017) Ocean deoxygenation, the global phosphorus cycle and the possibility of human-caused large-scale ocean anoxia. Philosophical Transactions of the Royal Society of A-Maths Physics Engineering Sciences, 375, p. 14. doi.org/10.1098/rsta.2016.0318.
- Wishner, K. F., et al. (2020) Ocean deoxygenation and copepods: Coping with oxygen minimum zone variability. Biogeosciences, 17(8), pp. 2315–2339. doi.org/10.5194/bg-17-2315-2020.