Inspired by earlier researchers, scientists have recently incorporated zinc (Zn) into indium sulfide (In2S3). They found that the synthesis allows this incorporation and enhances the stability of the resultant catalyst (ZnIn2S4). The research has been published in Nature Communications.
A fascinating means to decrease carbon dioxide (CO2) emissions and achieve carbon neutrality is the electrosynthesis of value-added fuels using CO2 as a feedstock. For the past 10 years, many active and selective catalysts have been studied for CO2 reduction reaction (CO2RR), and it is seen that CO and formate may be the only products that can achieve the industrialization trend of CO2RR in the near future.
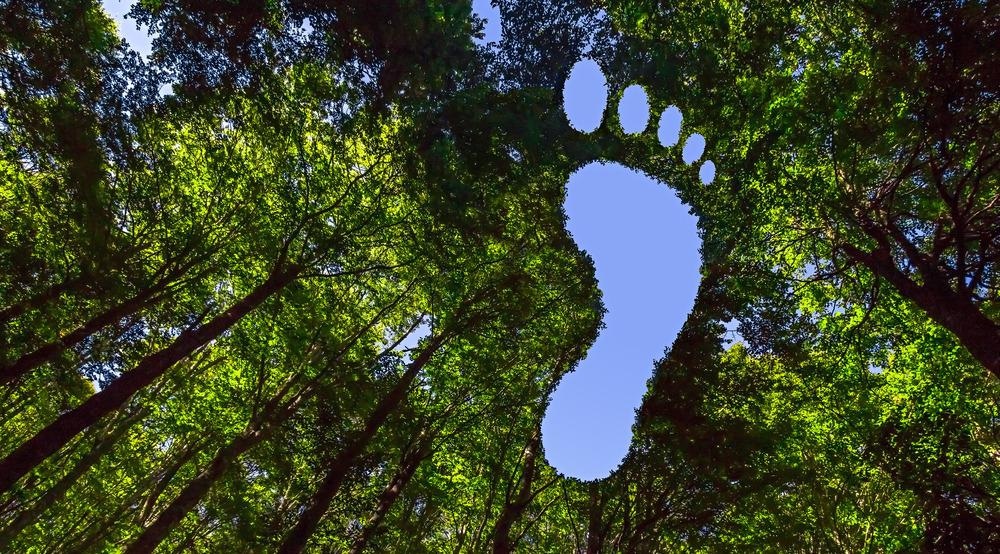
Image Credit: J.M. Image Factory/Shutterstock.com
Previous studies revealed that metals such as mercury, lead, bismuth (Bi), indium (In), tin, and cadmium, can convert CO2 to formate, however, most of these metals suffer from unsatisfactory selectivity or issues with toxicity.
This article details the incorporation of zinc (Zn) into indium sulfide (In2S3) synthesis which helps tune its phase and structure, improving the long-term stability of the resultant catalyst (ZnIn2S4). The catalyst morphology remains almost unchanged.
Results
Synthesis and Characterizations of Catalysts
Indium sulfide as a catalyst fascinated the researchers as S-doped In was demonstrated to be effective in catalyzing CO2RR to formate. The presence of S allows facile activation of H2O to form adsorbed H*, which reacts with absorbed CO2 to give HCOO* intermediates.
Results from earlier studies encouraged scientists to analyze the capability of indium sulfide instead of S-doped In in mediating CO2 to formate. Indium sulfide was produced hydrothermally by the reaction of InCl3·4H2O and C2H5NS in deionized water.
![Physical characterization of ZnIn2S4. a, b SEM images of the ZnIn2S4 catalyst. The right panel in b shows the crystal structure of ZnIn2S4. Scale bars, 5 µm (a) and 1 µm (b). c STEM-EDX elemental mapping of ZnIn2S4, exhibiting a uniform spatial distribution of Zn (red), In (green), and S (yellow), respectively. Scale bar, 1 µm. d, e Atomic-resolution Z-contrast images of ZnIn2S4 along [001] zone axis. Scale bars, 1 nm (d) and 0.5 nm (e). f The corresponding FFT pattern of (d). g The line intensity profile acquired along the yellow arrow in (d). h Atomic model of ZnIn2S4 along [001] zone axis. i–k XRD patterns (i), UPS spectra (j), and BET surface area analysis (k) of ZnIn2S4 and In2S3, respectively.](https://www.azocleantech.com/images/Article_Images/ImageForArticle_1345_1635754009087638.png)
Figure 1. Physical characterization of ZnIn2S4. a, b SEM images of the ZnIn2S4 catalyst. The right panel in b shows the crystal structure of ZnIn2S4. Scale bars, 5 μm (a) and 1 μm (b). c STEM-EDX elemental mapping of ZnIn2S4, exhibiting a uniform spatial distribution of Zn (red), In (green), and S (yellow), respectively. Scale bar, 1 μm. d, e Atomic-resolution Z-contrast images of ZnIn2S4 along [001] zone axis. Scale bars, 1 nm (d) and 0.5 nm (e). f The corresponding FFT pattern of (d). g The line intensity profile acquired along the yellow arrow in (d). h Atomic model of ZnIn2S4 along [001] zone axis. i–k XRD patterns (i), UPS spectra (j), and BET surface area analysis (k) of ZnIn2S4 and In2S3, respectively. Image Credit: Chi, et al., 2021.
Zn was incorporated into indium sulfide to enhance the stability of high-rate CO2RR. After synthesis, hexagonal-structured ZnIn2S4 (Figure 1i) microflowers were obtained. The thickness of the nanosheets obtained was ~8.69 nm for ZnIn2S4 and 9.32 nm for In2S3 as revealed through atomic force microscopy (AFM) measurements.
Energy-dispersive X-ray (EDX) spectrum elemental mapping showed a uniform spatial distribution of Zn, In, and S (Figure 1c).
High-angle annular dark-field scanning transmission electron microscopy (HAADF-STEM) was employed to analyze the detailed atomic structure of the ZnIn2S4. The fast Fourier transform (FFT) (Figure 1f) results show that Zn incorporation alters the coordination environment of indium sulfide and favors the electronic structure and catalytic properties.
Ultraviolet photoelectron spectroscopy (UPS) (Figure 1j) revealed a superior electronic property by the incorporation of the Zn element.
CO2RR Performances in a Flow Cell
The CO2RR properties of ZnIn2S4 and In2S3 catalysts were analyzed in a flow cell. Figure 2a shows the linear sweep voltammetry curves and Figure 2b depicts the Faradaic efficiency (FE) for formate.
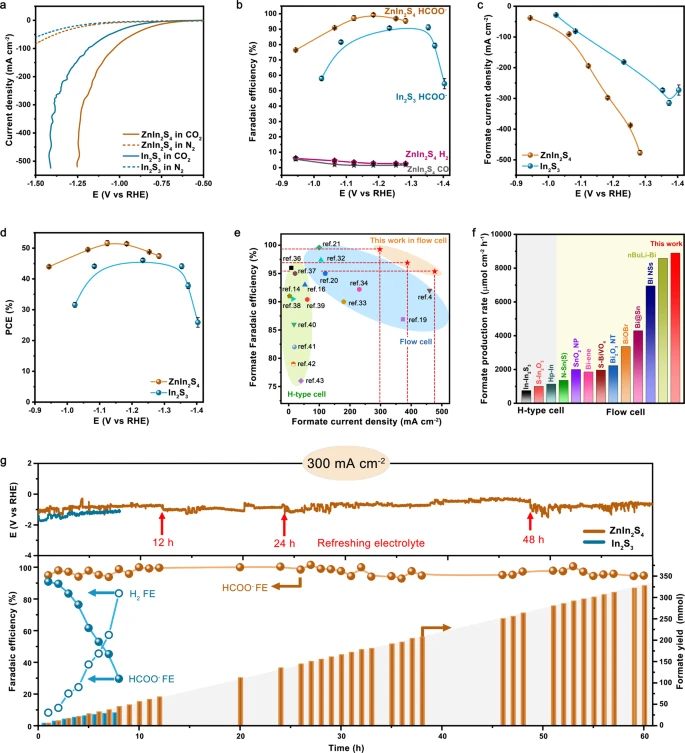
Figure 2. CO2RR performances. a, b The linear sweep voltammetry curves (a) and potential-dependent Faradaic efficiencies for products (b) on ZnIn2S4 and In2S3. c, d Partial current density (c) and half-cell PCE (d) for CO2-to-formate conversion on ZnIn2S4 and In2S3. e, f Comparison of formate partial current densities and FEs (e), and formate production rates (f) for various catalysts reported under KHCO3 environments. g Stability test of the ZnIn2S4 and In2S3 at 300 mA cm−2. The electrolyte was occasionally replaced by new 1 M KHCO3 solution (red arrows) to recover the ionic concentration and conductivity of the anolyte. The error bars represent the standard deviation of three independent measurements. Image Credit: Chi, et al., 2021.
Density functional theory (DFT) was employed to get insights into the CO2RR properties of the catalyst. The results showed that the S sites of ZnIn2S4 allow much smaller hydrogen adsorption free energy of 370 meV.
Comprehensive Stability Study
Figure 2g depicts the major finding that the CO2RR stability of indium sulfide can be remarkably improved by the incorporation of Zn.
Figure 3 depicts the multiple characterization techniques employed to analyze the structural evolution of ZnIn2S4 and In2S3 catalysts during CO2 electrolysis.
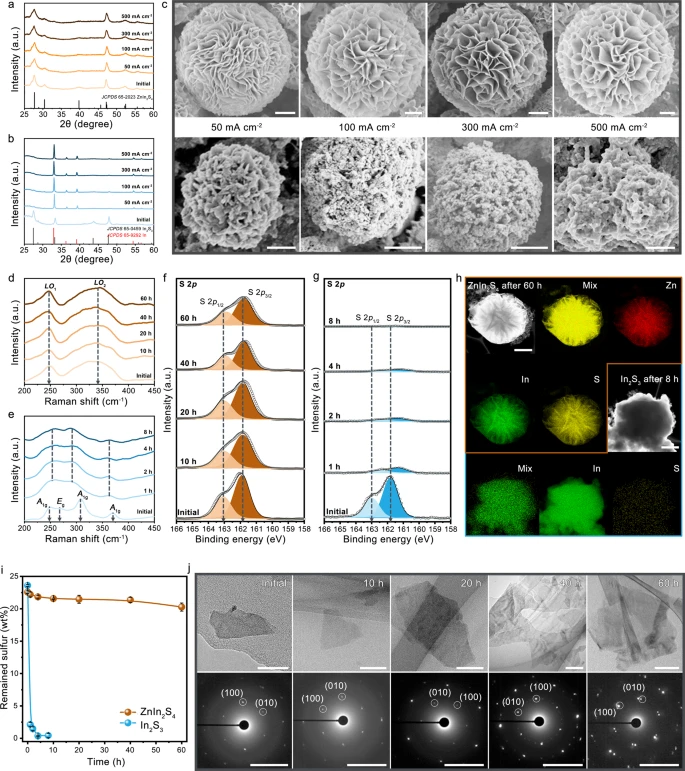
Figure 3. Structural stability of ZnIn2S4. a, b XRD patterns of ZnIn2S4 (a) and In2S3 (b) after CO2 electrolysis under various current densities for 10 min. c Corresponding SEM images of ZnIn2S4 (above) and In2S3 (bottom). Scale bars, 1 μm (above) and 500 nm (bottom). d–g Raman spectra of ZnIn2S4 (d) and In2S3 (e), and S 2p XPS spectra of ZnIn2S4 (f) and In2S3 (g) after CO2 electrolysis for various times at 300 mA cm−2. h STEM-EDX elemental mappings of ZnIn2S4 (scale bar: 1 μm) and In2S3 (scale bar: 600 nm) after running for 60 h and 8 h at 300 mA cm−2, respectively. i SEM-EDX measurements of the remained sulfur in catalysts after running for various times at 300 mA cm−2. The error bars represent the standard deviation of three independent measurements. j TEM (above, scale bars: 50 nm) and SAED patterns (down, scale bars: 5 1/nm) of ZnIn2S4 catalyst after CO2 electrolysis for various times at 300 mA cm−2. Image Credit: Chi, et al., 2021.
The amount of S left-back in ZnIn2S4 and In2S3 were quantified with SEM-EDX (Figure 3i) and it was noted that ZnS catalyst performed stably at high current densities. This is because of the strong interaction between Zn and S.
Stability Enhancement Mechanism
The obtained results indicate that the stability degradation of In2S3 is attributed to S leaching and that Zn as a stabilizer hinders S leaching.
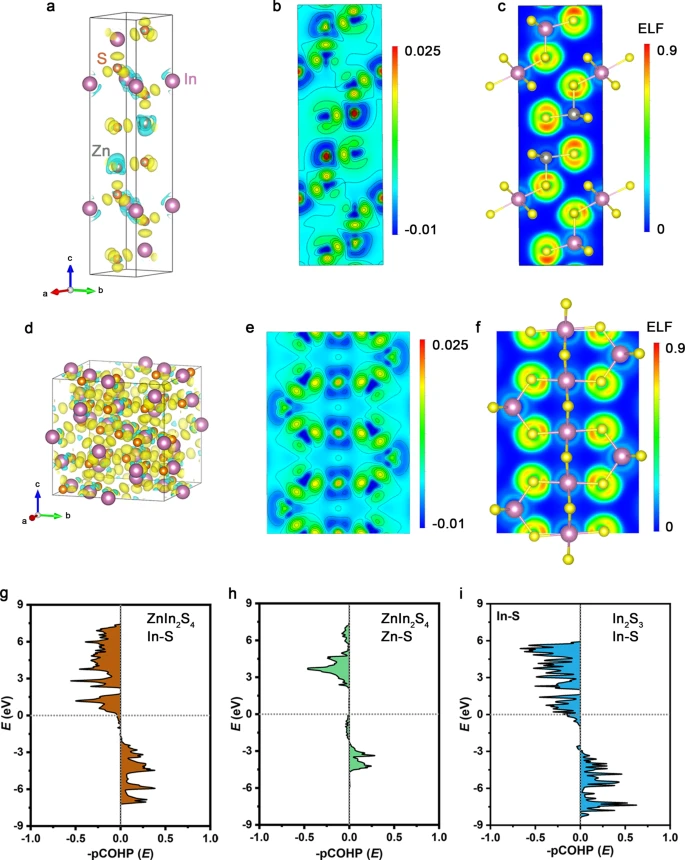
Figure 4. Enhanced covalency in ZnIn2S4. a, b Differential charge density (a) and projection on the (110) plane (b). c ELF of ZnIn2S4. d, e Differential charge density (d) and projection on the (011) plane (e). f ELF of In2S3. The azure and yellow clouds represent electron density depressions and accumulations, respectively. g–i COHPs for In−S bonding (g) and Zn−S bonding (h) of ZnIn2S4, as well as In−S bonding (i) of In2S3. Image Credit: Chi, et al., 2021.
The calculated results show that the bond breaking between In(Zn) and S in ZnIn2S4 is kinetically cumbersome elaborating the negligible S dissolution and thus exceptional long-term stability of the ZnIn2S4 catalyst.
Methodology
Material Synthesis
The chemicals Indium chloride tetrahydrate (InCl3·4H2O), thioacetamide (C2H5NS), and Zinc dichloride (ZnCl2) were used. In2S3 and ZnIn2S4 were synthesized using the same.
Material Characterizations
Characterization of the synthesized materials was carried out using XRD, STEM, HRTEM, SAED, and EDX elemental mapping.
Preparation of CO2RR Electrodes
The catalyst ink was produced by ultrasonic dispersion and the resultant ink was spread uniformly on the gas diffusion layer providing the prepared electrode with a catalyst loading of ~1.0 mg cm−2.
Electrochemical Measurements
Electrochemical measurements were carried out in a flow cell and gaseous CO2 (99.999%) was passed through the gas chamber. The CO2 electrolysis lasted for 10 minutes.
CO2RR Products Analysis
The gas product analysis was carried out with gas chromatography equipped with a thermal conductivity detector (TCD) to quantify H2 concentration and a flame ionization detector (FID) to analyze the CO content.
Quantification of formate products was carried out with the help of 1H NMR spectra measured with a Bruker 400 MHz spectrometer.
DFT Calculations
The DFT calculations were carried out by the Vienna ab initio simulation package (VASP) program with projector augmented wave (PAW) method.
Discussion
The article depicts a long-term formate electrosynthesis from CO2. The increased catalyst stability is due to the increase of In−S covalency, which hinders sulfur dissolution during CO2RR. The CO2-to-formate conversion was rapid and selective. The observations are anticipated to promote the creation of effective catalysts for commercial-scale electrosynthesis of formate.
Journal Reference:
Chi, L.-P., Niu, Z.-Z., Zhang, X.-L., Yang, P.-P., Liao, J., Gao, F.-Y., Wu, Z.-Z., Tang, K.-B., Gao, M.-R. (2021) Stabilizing indium sulfide for CO2 electroreduction to formate at high rate by zinc incorporation. Nature Communications, 12(5835). Available at: doi.org/10.1038/s41467-021-26124-y.
References and Further Reading
- Montoya, J. H., et al., (2017) Materials for solar fuels and chemicals. Nature Materials, 16, pp. 70–81. doi.org/10.1038/nmat4778.
- Mistry, H., et al. (2016) Nanostructured electrocatalysts with tunable activity and selectivity. Nature Reviews Materials, 1, p. 16009. doi.org/10.1038/natrevmats.2016.9.
- Gao, F.-Y., et al., (2020) High-curvature transition-metal chalcogenide nanostructures with a pronounced proximity effect enable fast and selective CO2 electroreduction. Angewandte Chemie International Edition, 59, pp. 8706–8712. doi.org/10.1002/anie.201912348.
- Fan, L., et al. (2020) Electrochemical CO2 reduction to high-concentration pure formic acid solutions in an all-solid-state reactor. Nature Communications, 11, p. 3633. doi.org/10.1038/s41467-020-17403-1.
- Chen, Z., et al., (2020) Grain-boundary-rich copper for efficient solar-driven electrochemical CO2 reduction to ethylene and ethanol. Journal of the American Chemical Society, 142, pp. 6878–6883. doi.org/10.1021/jacs.0c00971.
- Zhuang, T.-T., et al., (2018) Steering post-C–C coupling selectivity enables high efficiency electroreduction of carbon dioxide to multi-carbon alcohols. Nature Catalysis, 1, pp. 421–428. doi.org/10.1038/s41929-018-0084-7.
- Niu, Z.-Z., et al., (2021) Hierarchical copper with inherent hydrophobicity mitigates electrode flooding for high-rate CO2 electroreduction to multicarbon products. Journal of the American Chemical Society, 143, pp. 8011–8021. doi.org/10.1021/jacs.1c01190.
- Jiang, K., et al., (2018) Metal ion cycling of Cu foil for selective C–C coupling in electrochemical CO2 reduction. Nature Catalysis, 1, pp. 111–119. doi.org/10.1038/s41929-017-0009-x.
- Jouny, M., et al. (2018) General techno-economic analysis of CO2 electrolysis systems. Industrial & Engineering Chemistry Research, 57, pp. 2165–2177. doi.org/10.1021/acs.iecr.7b03514.
- Bushuyev, O. S., et al. (2018) What should we make with CO2 and how can we make it? Joule, 2, pp. 825–832. doi.org/10.1016/j.joule.2017.09.003.
- Hori, Y., et al. (1994) Electrocatalytic process of CO selectivity in electrochemical reduction of CO2 at metal electrodes in aqueous media. Electrochimica Acta, 39, pp. 1833–1839. doi.org/10.1016/0013-4686(94)85172-7.
- Ye, K., et al. (2020) In situ reconstruction of a hierarchical Sn−Cu/SnOx core/shell catalyst for high-performance CO2 electroreduction. Angewandte Chemie International Edition, 59, pp. 4814–4821. doi.org/10.1002/anie.201916538.
- Han, N., et al. (2018) Ultrathin bismuth nanosheets from in situ topotactic transformation for selective electrocatalytic CO2 reduction to formate. Nature Communications, 9, p. 1320. doi.org/10.1038/s41467-018-03712-z.
- Wang, Z., et al. (2020) Exfoliated ultrathin ZnIn2S4 nanosheets with abundant zinc vacancies for enhanced CO2 electroreduction to formate. ChemSusChem, 14, pp. 852–859. doi.org/10.1002/cssc.202002785.
- Zhou, J.-H., et al. (2019) Boosting electrochemical reduction of CO2 at a low overpotential by amorphous Ag-Bi-S-O decorated Bi0 nanocrystals. Angewandte Chemie International Edition, 58, pp. 14197–14201. doi.org/10.1002/anie.201908735.
- Ma, W., et al. (2019) Promoting electrocatalytic CO2 reduction to formate via sulfur-boosting water activation on indium surfaces. Nature Communications, 10, p. 892 doi.org/10.1038/s41467-019-08805-x.
- Grigioni, I., et al. (2020) CO2 electroreduction to formate at a partial current density of 930 mA cm−2 with InP colloidal quantum dot derived catalysts. ACS Energy Letters, 6, pp. 79–84. doi.org/10.1021/acsenergylett.0c02165.
- Zheng, X., et al. (2017) Sulfur-modulated tin sites enable highly selective electrochemical reduction of CO2 to formate, Joule, 1, pp. 794–805. doi.org/10.1016/j.joule.2017.09.014.
- Yang, J., et al. (2020) Bi-based metal-organic framework derived leafy bismuth nanosheets for carbon dioxide electroreduction. Advanced Energy Materials, 10, p. 2001709. doi.org/10.1002/aenm.202001709.
- Gong, Q., et al. (2019) Structural defects on converted bismuth oxide nanotubes enable highly active electrocatalysis of carbon dioxide reduction. Nature Communications, 10, p. 2807. doi.org/10.1038/s41467-019-10819-4.
- Cao, C., et al. (2020) Metal–Organic Layers Leading to Atomically Thin Bismuthene for Efficient Carbon Dioxide Electroreduction to Liquid Fuel. Angewandte Chemie International Edition, 59, pp. 15014–15020. doi.org/10.1002/anie.202005577.
- Wu, J., et al. (2016) Origin of the performance degradation and implementation of stable tin electrodes for the conversion of CO2 to fuels. Nano Energy. 27, pp. 225–229. doi.org/10.1016/j.nanoen.2016.06.028.
- Shi, Y., et al. (2020) Unveiling hydrocerussite as an electrochemically stable active phase for efficient carbon dioxide electroreduction to formate, Nature Communications, 11, p. 3415. doi.org/10.1038/s41467-020-17120-9.
- Cheng, H., et al. (2020) Surface nitrogen-injection engineering for high formation rate of CO2 reduction to formate. Nano Letters, 20, pp. 6097–6103. doi.org/10.1021/acs.nanolett.0c02144.
- Huang, Z.-F., et al. (2016) Hollow cobalt-based bimetallic sulfide polyhedra for efficient all-pH-value electrochemical and photocatalytic hydrogen evolution. Journal of American Chemical Society, 138, pp. 1359–1365. doi.org/10.1021/jacs.5b11986.
- Chai, B., et al. (2011) Template-free hydrothermal synthesis of ZnIn2S4 floriated microsphere as an efficient photocatalyst for H2 production under visible-light irradiation. Journal of Physical Chemistry C, 115, pp. 6149–6155. doi.org/10.1021/jacs.5b11986.
- Chen, Z., et al. (2009) Photocatalytic degradation of dyes by ZnIn2S4 microspheres under visible light irradiation. Journal of Physical Chemistry C, 113, pp. 4433–4440. doi.org/10.1021/jp8092513.
- Gou, X., et al. (2006) Shape-controlled synthesis of ternary chalcogenide ZnIn2S4 and CuIn(S,Se)2 nano-/microstructures via facile solution route. Journal of American Chemical Society, 128, pp. 7222–7229. doi.org/10.1021/ja0580845.
- Otsuka, R., et al. (2000) Thermoelectric properties of layer-structured (ZnS)mIn2S3. Key Engineering Materials. 181−182, pp. 59–62. doi.org/10.4028/www.scientific.net/KEM.181-182.59.
- Aymerich, F., et al. (1979) Electron band structure of α-ZnIn2S4 and related polytypes. Solid State Communications, 29, pp. 235–238 doi.org/10.1016/0038-1098(79)91045-7.
- Fu, X., et al. (2010) Photocatalytic performance of tetragonal and cubic β-In2S3 for the water splitting under visible light irradiation. Applied Catalysis B: Environmental, 95, pp. 393–399. doi.org/10.1016/j.apcatb.2010.01.018.
- Ma, W., et al. (2021) Monoclinic Scheelite Bismuth Vanadate derived Bismuthene nanosheets with rapid kinetics for electrochemically reducing carbon dioxide to formate. Advanced Functional Materials, 31, p. 2006704. doi.org/10.1002/adfm.202006704.
- García de Arquer, F. P., et al. (2018) 2D metal oxyhalide-derived catalysts for efficient CO2 electroreduction. Advanced Materials, 30, p. 1802858 doi.org/10.1002/adma.201802858.
- Xing, Y., et al. (2020) Bi@Sn core–shell structure with compressive strain boosts the electroreduction of CO2 into formic acid. Advanced Science, 7, p. 1902989. doi.org/10.1002/advs.201902989.
- Liang, C., et al. (2018) High efficiency electrochemical reduction of CO2 beyond the two-electron transfer pathway on grain boundary rich ultra-small SnO2 nanoparticles. Journal of Materials Chemistry A, 6, pp. 10313–10319. doi.org/10.1039/C8TA01367E.
- Shang, H., et al. (2020) Design of a single-atom indiumδ+-N4 interface for efficient electroreduction of CO2 to formate. Angewandte Chemie International Edition, 59, pp. 22465–22469. doi.org/10.1002/anie.202010903.
- Kwon, I. S., et al. (2019) Selective electrochemical reduction of carbon dioxide to formic acid using indium–zinc bimetallic nanocrystals. Journal of Materials Chemistry A, 7, pp. 22879–22883. doi.org/10.1039/C9TA06285H.
- Zhang, X., et al. (2020) Defects and conductive nitrogen−carbon framework regulated ZnInOx nanosheets for boosting CO2 electrocatalytic reduction. Applied Catalysis B: Environmental, 279, p. 119383. doi.org/10.1016/j.apcatb.2020.119383.
- Luo, W., et al. (2019) 3D hierarchical porous indium catalyst for highly efficient electroreduction of CO2. Journal of Materials Chemistry A, 7, pp. 4505–4515. doi.org/10.1039/C8TA11645H.
- Zhang, A., et al. (2019) Harmonizing the electronic structures of the adsorbate and catalysts for efficient CO2 reduction. Nano Letters, 19, pp. 6547–6553 doi.org/10.1021/acs.nanolett.9b02782.
- Wang, J., et al. (2019) Heterostructured intermetallic CuSn catalysts: high performance towards the electrochemical reduction of CO2 to formate. Journal of Materials Chemistry A, 7, pp. 27514–27521. doi.org/10.1039/C9TA11140A.
- Zhang, A., et al. (2018) Nickel doping in atomically thin tin disulfide nanosheets enables highly efficient CO2 reduction. Angewandte Chemie International Edition, 57, pp. 10954–10958. doi.org/10.1002/anie.201806043.
- Yuan, X., et al. (2020) Decoration of In nanoparticles on In2S3 nanosheets enables efficient electrochemical reduction of CO2. Chemical Communications, 56, pp. 4212–4215. doi.org/10.1039/C9CC10078D.
- Shen, S., et al. (2011) Microwave-assisted hydrothermal synthesis of transition-metal doped ZnIn2S4 and its photocatalytic activity for hydrogen evolution under visible light. Journal of Power Sources, 196, pp. 10112–10119. doi.org/10.1016/j.jpowsour.2011.08.103.
- Lopez-Rivera, S. A., et al. (1995) Raman study of a ZnIn2S4 layered compound. Semiconductor Science and Technology, 10, pp. 645–652. doi.org/10.1088/0268-1242/10/5/013.
- Tao, H., et al. (2006) Raman scattering studies of the Ge–In sulfide glasses. Solid State Communications, 137, pp. 408–412. doi.org/10.1016/j.ssc.2005.12.032.
- RRUFFTM Project. Available at: https://rruff.info/Indium.
- Wu, Y., et al. (2021) Converting copper sulfide to copper with surface sulfur for electrocatalytic alkyne semi-hydrogenation with water. Nature Communications, 12, p. 3881. doi.org/10.1038/s41467-021-24059-y.
- Hu, C., et al. (2017) In situ electrochemical production of ultrathin nickel nanosheets for hydrogen evolution electrocatalysis. Chem, 3, pp. 122–133 doi.org/10.1016/j.chempr.2017.05.011.
- Luc, W., et al. (2019) SO2 induced selectivity change in CO2 electroreduction. Journal of American Chemical Society, 141, pp. 9902–9909. doi.org/10.1021/jacs.9b03215.
- Liu, D., et al. (2020) Understanding how atomic sulfur controls the selectivity of the electroreduction of CO2 to formic acid on metallic Cu surfaces. Journal of Physical Chemistry C, 124, pp. 6145–6153. https://doi.org/10.1021/acs.jpcc.9b11830.
- Aymerich, F., et al. (1980) Electronic properties of the layer compound ZnIn2S4. Physica B+C, 99, pp. 314–317. doi.org/10.1016/0378-4363(80)90252-1.
- Tao, W., et al. (2020) Electronic structure and oxidation mechanism of nickel–copper converter matte from first-principles calculations. ACS Omega, 5, pp. 20090–20099 doi.org/10.1021/acsomega.0c01713.
- Wei, T.-R., et al. (2020) Exceptional plasticity in the bulk single-crystalline van der Waals semiconductor InSe. Science, 369, pp. 542–545. doi.org/10.1126/science.aba9778.
- Hao, Y.-C. et al. (2019) Promoting nitrogen electroreduction to ammonia with bismuth nanocrystals and potassium cations in water. Nature Catalysis, 2, pp. 448–456. doi.org/10.1038/s41929-019-0241-7.
- Ma, M., et al. (2020) Role of ion-selective membranes in the carbon balance for CO2 electroreduction via gas diffusion electrode reactor designs. Chemical Science, 11, pp. 8854–8861. doi.org/10.1039/D0SC03047C.
- Kuhl, K. P., et al. (2012) New insights into the electrochemical reduction of carbon dioxide on metallic copper surfaces. Energy & Environmental Science, 5, pp. 7050–7059. doi.org/10.1039/C2EE21234J.
- Kresse, G & Hafner, J (1993) Ab initio molecular dynamics for liquid metals. Physical Review B, 47, pp. 558–561. doi.org/10.1103/physrevb.47.558.
- Blöchl, P. E., (1994) Projector augmented-wave method. Physical Review B, 50, pp. 17953–17979. doi.org/10.1103/PhysRevB.50.17953.
- Perdew, J. P., et al. (1996) Generalized gradient approximation made simple. Physical Review Letters, 77, pp. 3865–3868. doi.org/10.1103/PhysRevLett.77.3865.
- Yoo, J. S., et al. (2016) Theoretical insight into the trends that guide the electrochemical reduction of carbon dioxide to formic acid. ChemSusChem, 9, pp. 358–363. doi.org/10.1002/cssc.201501197.
- Jiang, B., et al. (2018) Boosting formate production in electrocatalytic CO2 reduction over wide potential window on Pd surfaces. Journal of American Chemical Society, 140, pp. 2880–2889. doi.org/10.1021/jacs.7b12506.
- Maintz, S., et al. (2016) LOBSTER: a tool to extract chemical bonding from plane-wave based DFT. Journal of Computational Chemistry, 37, pp. 1030–1035 doi.org/10.1002/jcc.24300.
- Dronskowski, R & Bloechl, P E (1993) Crystal orbital Hamilton populations (COHP): energy-resolved visualization of chemical bonding in solids based on density-functional calculations. Journal of Physical Chemistry, 97, pp. 8617–8624. doi.org/10.1021/j100135a014.
- Deringer, V. L., et al. (2011) Crystal orbital Hamilton population (COHP) analysis as projected from plane-wave basis sets. Journal of Physical Chemistry A, 115, pp. 5461–5466. doi.org/10.1021/jp202489s.